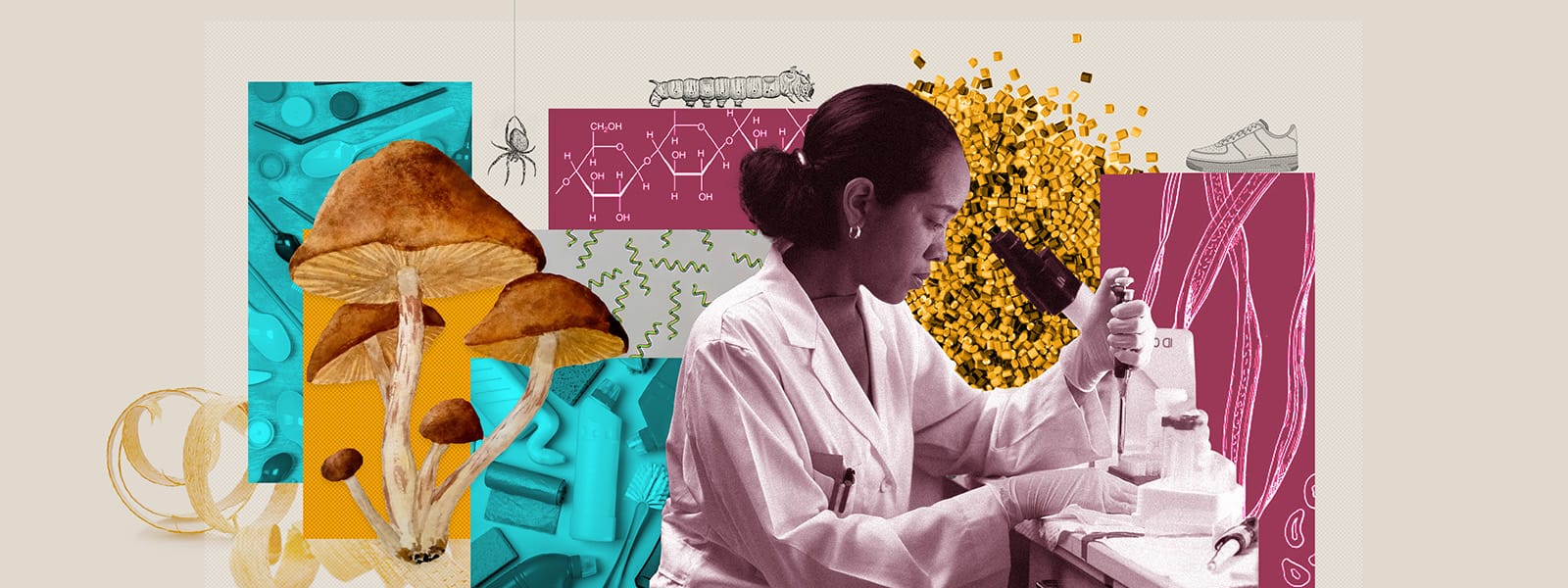
This story was originally published by Knowable Magazine.
Materials scientists are cooking up environmentally friendly polymers from natural sources like silk, plant fibers and whole algae. Economics and acceptance remain hurdles.
It was hailed as a wonderful thing: During the oil boom in the 1950s, chemists began to render the waste coming out of refineries into plastic — plastic packaging, plastic furniture, plastic fibers woven into synthetic cloth. These were miracle materials, moldable and pliable but strong and lasting. In the decades since, annual global plastic production has skyrocketed: Humans have created 8 billion metric tons of plastic.
That boom has, to put it lightly, brought problems. More than half the plastic ever produced —some 5 billion metric tons — lies smeared across the surface of the Earth. Every day, more than 10,000 metric tons of plastic wash into the oceans. Plastic’s durability, one of the properties that makes this material so miraculous, has rendered it a potent pollutant.
To be fair to the early boosters, plastics have changed the world. So many essential technologies — from motor vehicles to cell phones to computers — use plastic. Foam insulation has helped to make homes 200 times more energy efficient. Plastic films extend the shelf life of perishable foods.
“I don’t like how people demonize plastics as if it is the most evil thing we have ever made,” says Eleftheria Roumeli, a physicist at the University of Washington and coauthor of a 2023 examination of sustainable polymers in the Annual Review of Materials Research. “It is a product of brilliant engineering.”
Rather than abandon this material, she thinks, we need to find a better, kinder version — polymers with the tensile strength and flexibility of modern plastics that are derived from sustainable biological sources and can be effectively returned to the environment.
This means rethinking plastic production from the ground up.
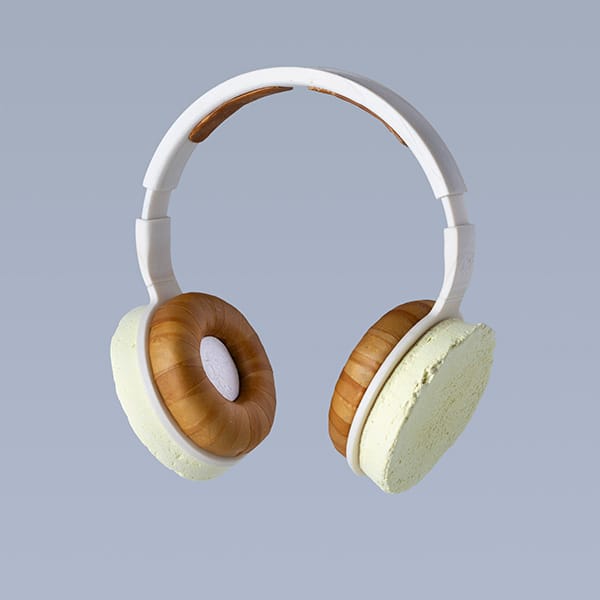
From monomer to polymer
The current approach to plastic production consists of two big steps: first a breaking down, then a building back up.
The breaking down — “cracking,” as it’s known, conducted under high heat and pressure — turns refined petroleum materials into simple molecules known as monomers. These become the backbone of the product that’s built back up. The chains or lattices that result are known as polymers and serve as the basic structural component of any plastic.
But the plastic is not done yet. Next comes the incorporation of additives — colorants and flame retardants and fillers. Materials scientists consider a wide variety of variables, from “hardness” to “tear strength” to “tensile modulus,” that indicate how a plastic fares under different kinds of stresses. The most important additives tune these properties, generally by tweaking the bonds between polymer chains. Chemicals known as plasticizers, for instance, embed themselves between chains, helping to increase flexibility — but, as a tradeoff, making the plastic easier to tear apart.
By mixing and matching polymers and additives, chemists create the final composite materials that are used in food wrappers and soda bottles, as microbeads in cosmetics, even as the flexible hydrogels that, in the form of contact lenses, we affix to our corneas to sharpen our sight. Through chemistry, a single polymer like polyvinyl chloride — or PVC, as it’s often known — can be rendered into rigid stormwater piping or clothing.
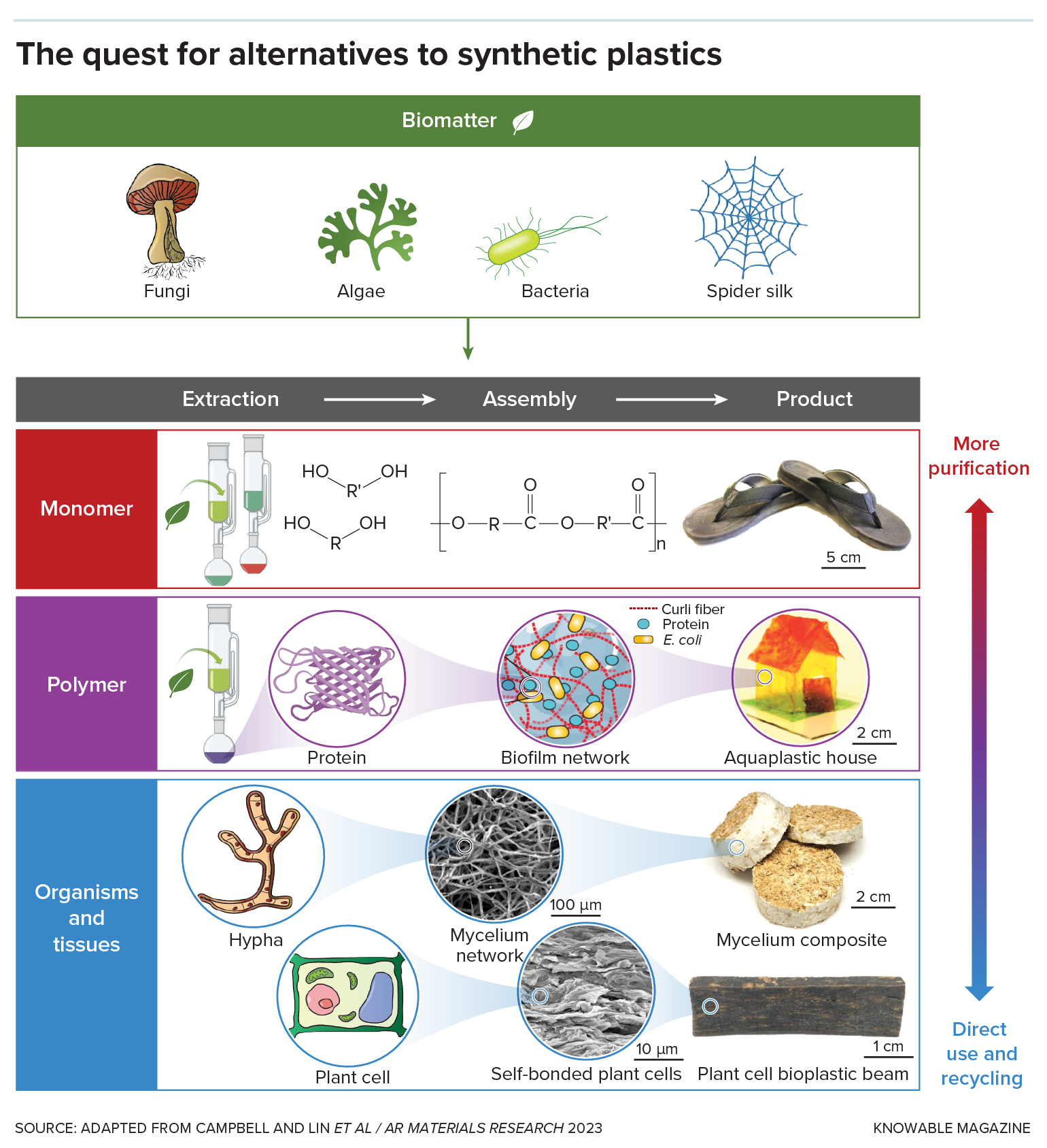
Plastic production accounts for as much as 8 percent of global fossil fuel consumption — a figure that could rise, according to one estimate, to 20 percent by 2050. But chemists were creating “synthetic” plastics decades before the oil industry took off, from, among other materials, waste oat husks and vegetable oil. One of the tacks toward more sustainable plastics is to turn back to such biological sources.
In 2006, for example, the Brazilian petrochemical company Braskem launched experiments to see if they could economically render sugar into ethylene, the most important monomer in commodity plastic production. By 2010, Braskem was selling a “fully biological” polyethylene plastic, or bio-PE.
The big upside of this material is that sugarcane will sequester carbon from the atmosphere as it grows. And since, structurally speaking, bio-PE is indistinguishable from its synthetic twin, bio-PE has been easy to deploy in applications such as food packaging, cosmetics and toys.
But being chemically indistinguishable is also a problem. Since polyethylene does not appear in natural environments, few microbes have developed the ability to break its molecular bonds. Bio-PE therefore does nothing to solve the waste problem. Just because something is a “bioplastic,” in other words, does not mean it is inherently sustainable.
“None of these terms are sufficiently regulated or meaningfully defined, which causes a lot of confusion,” says Rachel Meidl, a fellow in energy and sustainability at Rice University's Baker Institute.
Meidl categorizes plastics and their potential substitutes as existing across four quadrants. One axis charts the source of the materials: Some are “biobased,” drawn from biological materials, while some are petroleum-based. The other axis maps the downstream fate: Some materials are biodegradable and some are not. But even a material that lands in the best of these quadrants — both bio-based and biodegradable — is not necessarily a panacea. Being biodegradable just means that a material can be broken apart by microbes, even if the result is small bits of microplastics. The ideal materials are not just biodegradable but also compostable — a narrower category that indicates the material can break down into organic components that are harmless to plants and animals.
Compostability, unfortunately, is not easily achieved. You’ve almost certainly encountered polylactic acid, or PLA, in the form of compostable cutlery and take-out containers. The most common biobased plastic, PLA is technically compostable, but under specific conditions obtained only in industrial facilities that do not yet exist in sufficient numbers. Since most of today’s PLA take-out containers wind up discarded alongside food scraps, composters must waste time sorting out the two.
One way to improve plastics might be to search for better bio-sourced monomers. In 2020, a team of scientists in California reported they had isolated a type of monomer called a polyol from algae-produced oils, then reassembled it into a foam-like plastic that could be used in commercial footwear. The material effectively degraded when placed in soils.
Some scientists, though, think a better choice is to leave behind the standard energy-intensive two-step process of breaking down to monomers and then building back up. The natural world already supplies promising polymers that are all compostable, says David Kaplan, a biomedical engineer at Tufts University. And since they degrade at different timescales, if you select the right polymer or tune it properly, you can create materials to suit varied applications.
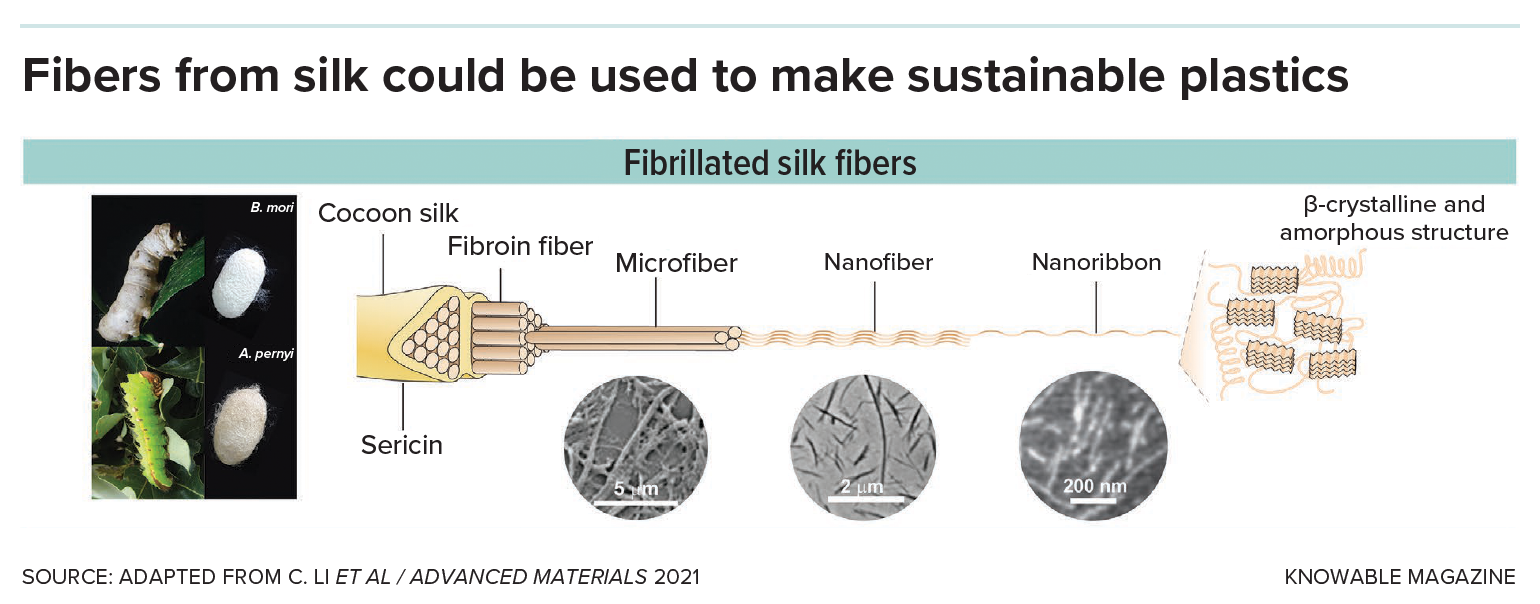
Consider cellulose, the most common biological polymer, present in the cell walls of plants. It is essentially a chain of sugar molecules, but these chains are assembled into tiny threads called nanofibrils, which are bundled into microfibers and then into the large fibers that are visible, such as the stringy strands in celery, for example. Materials scientists call this a hierarchical structure.
Synthetic polymers, in contrast, typically are pressed into a hopper and extruded into a homogenous glob. The result is “strong, hard bonds” between molecules, Kaplan says. “Biology doesn’t do much of that.” Instead, biopolymers feature much weaker bonds — typically electrostatic interactions that link the hydrogen atoms in one polymeric molecule to those in another, but at very high densities.
But by better understanding these structures, engineers will be able to improve upon the biological materials. Research has shown, for example, that the thinner a cellulose fiber, the greater the tensile strength, which means the material resists breaking under tension. The increased surface area means hydrogen atoms are better positioned to dynamically create and break bonds between adjoining chains.
Going straight to cells
Once you’ve given up monomers — scratching out an entire step in the plastic production process — why not go further? Some materials scientists are pursuing what Kaplan calls “bottom-up design”: Rather than isolating and remaking individual biopolymers, they use what nature has provided — creating bioplastic materials from whole cells or other biological materials, no breaking open and extraction required.
Roumeli, for example, has mined the promise of algal cells. They’re small, and therefore easily manipulable; they contain large amounts of proteins, which are biological polymers, alongside other useful materials. She and her students took powdered algae and passed it through a hot presser. After several trials in which they varied the pressing time, temperature and the amount of pressure applied — all of which affect the way the molecules bond — they found they could produce a material that was stronger than many commodity plastics.
The material was also recyclable: It could be ground back to powder and pressed again. (The tests showed that the material lost some strength with each generation of recycling, which is also the case for synthetic plastics.) If it were to be carelessly tossed into the dirt, the material would break apart at the same rate as a banana peel.
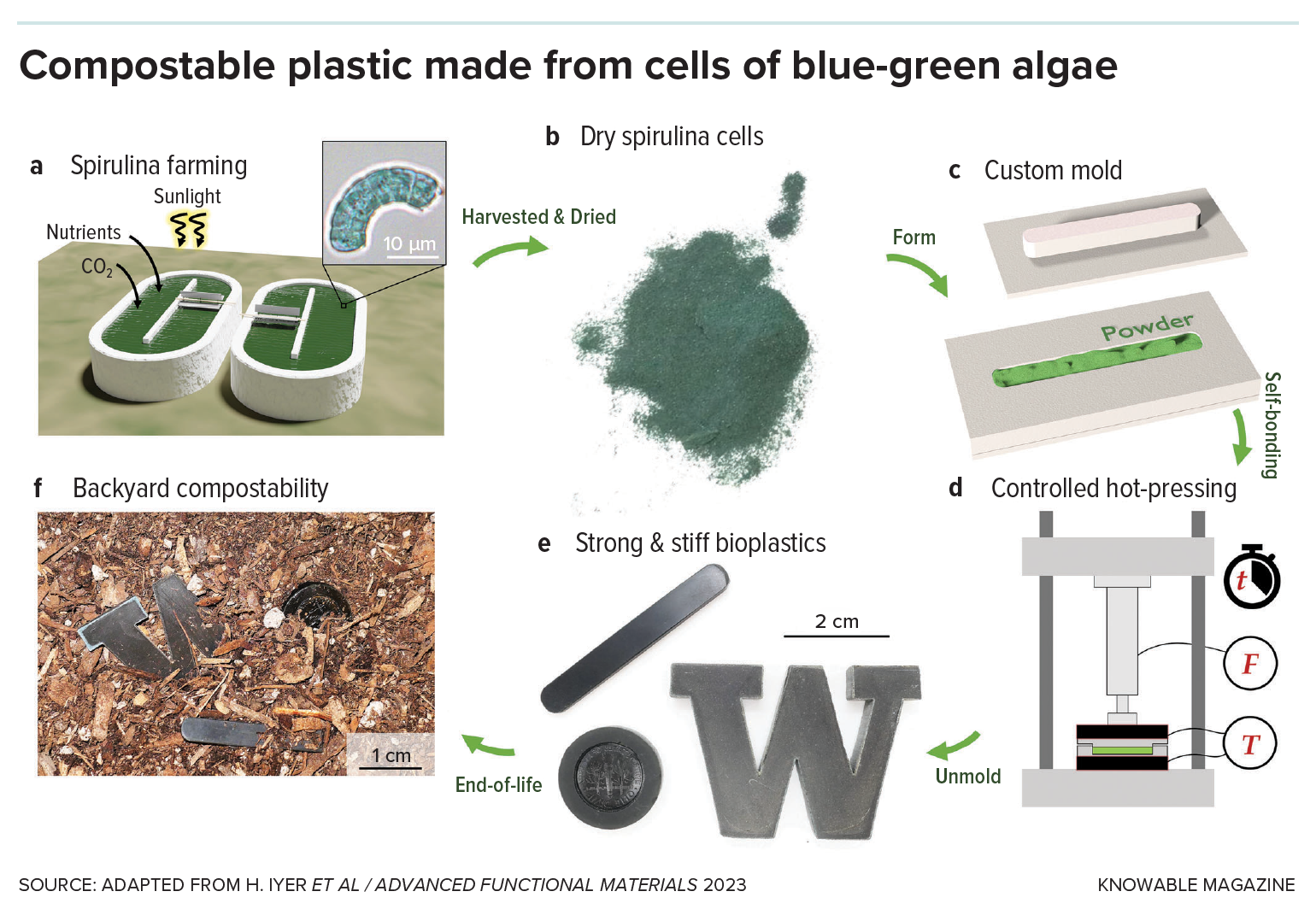
Kaplan has conducted similar work with silk, long presumed too fragile to be thermally processed. The thought was that the hydrogen bonds would break down under heat and the silk would simply burn. But in a 2020 paper, Kaplan and colleagues demonstrated that pellets of silk could be molded, like plastic, into a tunable material. Since then, he’s found that entire cocoons can be processed this way.
Such materials are a win-win, Roumeli says. They’re renewable and free of fossil fuels and can even soak up atmospheric carbon as they grow. They could biodegrade completely. “The only thing that is not a win is our economics — and our scalability,” she says.
Here is perhaps the biggest problem with this new approach to plastics: Its radical nature means that it’s going to be expensive, at least for now. To make a product cheap, you want to be able to produce it in already existing facilities, since that helps a startup avoid substantial capital costs. But the owners of those facilities are liable to see biological composites as too impure — as “junk,” says Gadi Rothenberg, a chemist at the University of Amsterdam’s Van ‘t Hoff Institute for Molecular Sciences.
Rothenberg notes that in the feedstock used to produce polyethylene terephthalate, the plastic used in soda bottles, only one molecule out of every 100,000 is anything but the desired monomer. Biological materials are rarely so pure.
Manufacturers may also simply prefer to go with tried-and-true over the unexpected. Rothenberg developed his own, plant-based sustainable polymer, which he figured was close enough to standard materials to be an easy, “drop-in” choice for furniture production. But when he first took it to companies, “in the beginning, they didn’t even want to hear about it,” he says. Even bio-PE, the sugarcane-based product that is chemically identical to its synthetic relative, costs as much as 30 percent more to manufacture, according to some figures, and so companies concerned about the bottom line are going to stick with the incumbent.
Today, bio-based plastics make up less than 1 percent of the current market, according to the trade association Plastics Europe. The push for biobased polymers “is not going to go anywhere until it reaches par on economics,” Rothenberg says. Ultimately, he predicts, governments will have to recognize the true, full cost of traditional plastic — its carbon footprint and the expenses involved with cleaning up pollution — before more sustainable materials will take hold.
Scientists in the vanguard are hopeful, though. Roumeli notes that synthetic polyethylene — “the cheapest and most produced and most consumed plastic that we have today” — once was a novelty. Kaplan says he has no doubt that one day, “all these precursors and polymers will be made biologically, or with true circularity in mind.”
“But we’re not there yet,” he adds. The trouble is, with plastic waste accumulating and temperatures rising, we may not have much time to wait.
First published in Knowable Magazine. You can read the article here.
Boyce Upholt is a freelance writer based in New Orleans. He writes the Substack Southlands, and his first book, The Great River: The Making and Unmaking of the Mississippi, will be published by W.W. Norton in 2024.
If you liked what you just read and want more of Our Brew, subscribe to get notified. Just enter your email below.
Related Posts
Pope Leo Xiv is the First Member of the Order of St. Augustine to Be Elected Pope – but Who Are the Augustinians?
Jun 04, 2025
People Say They Prefer Stories Written by Humans Over AI-generated Works, Yet New Study Suggests That’s Not Quite True
Apr 02, 2025
What are AI Hallucinations? Why AIs Sometimes Make Things Up
Mar 25, 2025